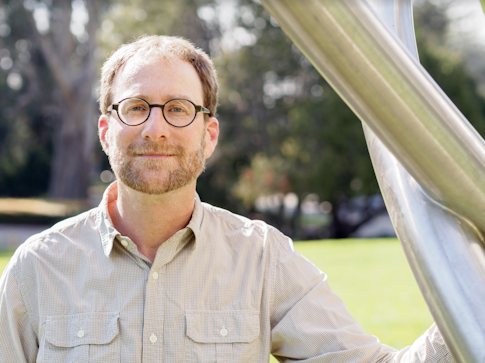
Dan Feldman is a professor in the Department of Molecular & Cell Biology at the University of California, Berkeley. His lab studies the somatosensory cortex in rodents, which is famous for its organized groupings of neurons, called ‘barrels,’ that receive inputs carrying information from specific facial whiskers. Feldman’s lab uses this brain region as a place to explore core principles of information processing by cortical circuits.
In 2014, he received a SFARI Explorer Award (and, one year later, a SFARI Pilot Award) to assess cortical circuitry in different genetic mouse models of autism. Specifically, the lab was interested in testing an influential idea about autism: the excitatory-inhibitory (E-I) imbalance hypothesis. This proposes that an excess of excitatory signaling relative to inhibitory signaling in the brain leads to symptoms of autism.
Using four disparate genetic mouse models of autism (Fmr1, Cntnap2, Tsc2 and 16p11.2 deletion mice), Feldman’s lab found evidence for the E-I imbalance hypothesis, but not exactly in the way many expected, since the changes appeared to reflect a compensation for some other problem in the circuit, rather than a primary deficit causing circuit hyperexcitability1.
I recently spoke with Feldman to discuss these findings and what they might mean for therapeutic approaches aimed at restoring inhibition in autism. The interview has been edited for clarity and brevity.
You’ve been studying somatosensory cortex for some time to understand basic cortical processing. What motivated you to study it in the context of autism?
It had always been a goal to understand enough about cortical circuits and cortical information processing to be able to use that knowledge to somehow make a positive impact on disease. But honestly, I wasn’t really tied to any one particular brain disorder. Then, in reading papers about autism, it seemed that the E-I imbalance hypothesis — which had been proposed by Rubenstein and Merzenich2 — was exactly the kind of hypothesis that we could test very well using the physiology approaches that we were pretty good at. At that same time, SFARI was reaching out broadly to groups that weren’t doing autism research yet but were interested in applying various techniques to that problem. So it seemed like a natural match.
We’re looking at somatosensory cortex, not because we think it’s the root of all autism, but because we think that, if autism really stems from problems in brain development, then circuit dysfunction will occur relatively broadly across cortical areas. Plus, it has been estimated that 85 percent of people with autism have some kind of sensory abnormality, and it’s typically either auditory or tactile disturbances, and very often it’s hypersensitivity.
How would you describe the E-I ratio hypothesis of autism?

The E-I ratio hypothesis has two components basically. The first component is at the synaptic level. This states that there is either too much excitation or too little inhibition, upsetting the balance of synaptic currents flowing in cells, so there’s a greater ratio of excitatory current (E) to inhibitory current (I). Strictly speaking, the disruption is thought to be in the ratio of excitatory to inhibitory synaptic conductance, which is the opening of neurotransmitter-gated channels that allows current to flow.
The second component is at the level of neural excitability, depolarization and spiking. The idea is that if neurons are in the business of integrating E and I in order to depolarize and spike, then if the E-I ratio goes up, synaptic responses would be more depolarizing, and therefore, cells will spike more. This extra spiking is hypothesized to constitute noise in brain circuit computations, which could explain some of the cognitive defects in autism. In addition, these excess spikes might lead to increased seizure risk, which is associated with some forms of autism. So, there are these two levels of the hypothesis: Does E-I ratio change, and does that actually cause more synaptic depolarization and more spiking?
The E-I ratio hypothesis is exciting because it could, in principle, represent a common circuit impairment that is shared across multiple forms of autism, including multiple genetic forms that can be modeled in transgenic mice. And if this is true, it suggests potential therapies that could be broadly effective across forms of autism. This is one reason it has garnered so much attention.
What was the status of experimental evidence regarding this hypothesis prior to your study?
Many papers have tested aspects of this hypothesis over the years, and many of them report a deficit in synaptic inhibition, suggesting the hypothesis may be correct. But other findings have raised questions. For example, in some mouse models of autism, it was found that excitation dropped or the E-I ratio dropped, which goes in the opposite direction of the original hypothesis. This led some to expand the hypothesis to propose any change in the E-I ratio — up or down — is deleterious and leads to autism.
Other groups proposed that even if synaptic properties don’t change, if the overall firing rate of excitatory neurons is increased in some brain area, or the firing rate of inhibitory neurons is decreased, then that is evidence for the E-I ratio going up. In this reformulation, the hypothesis has wandered far away from its roots and has become very hard to prove or disprove.
“We struggled for a while to figure out how an increase in E-I ratio could be associated with no change in synaptic depolarization or spiking.”
Another problem is evaluating whether E-I changes are common across brain areas and genetic forms of autism. Virtually all studies focus on a given lab’s one favorite transgenic mouse model and looked in one favorite brain area, at one favorite cell type. Different transgenic mice have been assessed in separate studies, looking in different brain areas, and using different physiology methods. But really, to test the idea that this hypothesis can explain common dysfunction across different genetic types of autism requires a more systematic approach, one where you use the same physiology method, same cell type, same brain area, to test for common changes in E or I or in E-I ratio.
Finally, while many papers in the literature tested whether E or I changed at the synaptic level, there were few studies testing whether circuit activity and spike probability were abnormal — the second component of the hypothesis. So we thought we would try to assess both of these aspects at once to see whether this hypothesis held up.
And what did you find?

We chose four well-validated mouse models of autism, in which the mutations occur in completely different genetic pathways, so it wasn’t clear that they would all show the same phenotype. Yet what we found was that, across all the models, there actually was a common decrease in feedforward inhibition in somatosensory cortex. And it was associated with a smaller and more variable decrease in excitation across all the models. This confirmed the first component of the hypothesis — E-I ratio really does go up.
We then tested the second component: Does that lead to more synaptic depolarization and more spiking? And here, we were very surprised to see that peak depolarization actually didn’t go up in any of the mouse lines. We struggled for a while to figure out how an increase in E-I ratio could be associated with no change in synaptic depolarization or spiking.
To explain it, we had to go back to details about how synaptic integration works. The hypothesis says that if the E-I ratio stays the same, cell excitability will be normal, but if E-I ratio goes up, cells will depolarize more and spike more. But actually that’s not necessarily true. That’s because the current that actually flows through these open excitatory or inhibitory channels depends on the resting potential of a cell. At a neuron’s resting potential, there’s a lot more driving force on excitation than inhibition. So if E and I conductance both dropped by the same proportion — preserving the E-I ratio — the decrease in E will have a bigger impact than the decrease in I, and net synaptic depolarization would actually go down, not stay the same.
“Compensation is part of the brain’s natural endogenous toolbox to stabilize firing rate.”
So to understand how a change in E or I translates into a synaptic potential, driving force needs to be taken into account.
Right. And if both E and I are reduced, one way to boost synaptic potentials back to normal is to decrease I a bit further, which would give you a slight increase in E-I ratio. This is what we found in all four of our mouse mutants. In each cell, I went down more than E, but by an amount that was exactly calibrated to stabilize the peak synaptic depolarization. So that makes it really seem like these E-I changes might be compensatory to stabilize synaptic function and spike rate, rather than to drive excess spiking. In the last part of our study, we measured spike rate in vivo in three of these mouse lines and found that spiking was normal and in one case reduced, but never increased. This is consistent with E-I changes being compensatory in this brain area.
Compensation is part of the brain’s natural endogenous toolbox to stabilize firing rate. It’s very important to the cortex to have a firing rate within a workable functional range. The brain has multiple homeostatic mechanisms to maintain this, and adjusting E-I ratio is one of them. Indeed, in a prior study3, we found that sensory manipulations in wild-type mice adjust E-I ratio in the exact quantitative way that we found in the autism mouse lines. So this leads to the hypothesis that the E and the I and the E-I ratio changes we observed in these autism models do not represent the direct effects of the gene mutations. They may instead represent a common, natural compensation to whatever the initial circuit problem was.
Couldn’t a natural compensation still disrupt circuit function?
Yes, absolutely. I can imagine a few ways that compensation could relate to autism. One possibility is that the compensation is perfect. Cortical function, at least in the somatosensory cortex, works just fine. In this case, somatosensory cortical function is just not relevant for autism; it’s some problem somewhere else in the brain.
Another explanation could be that compensation occurs, but it’s insufficient. So maybe it largely restores firing rates, but maybe it’s not perfect. Small residual differences may be enough to cause the cognitive and behavioral differences observed in autism. While we found that the firing rate in the somatosensory cortex appeared normal under controlled, simple, quiet conditions, it is possible that in a more challenging environment — maybe when the animal is stressed or when there’s intense sensory activity, maybe in brain areas that naturally have denser codes — maybe these mechanisms fail, and parts of the brain generate too many spikes under those conditions.
Then the third possibility is that compensation is successful at normalizing the firing rate because that’s so essential for cortical function, but it has a maladaptive byproduct of impairing something else about cortical coding or cortical computation. It could be that something else is causing a degradation in information processing and is leading to the autism features.
What are your next steps with this?
We’re going to try to address the question of how information processing within brain circuits is altered in autism. Think about spikes propagating through a network. Those spikes carry information by their pattern and by which neurons they involve. What we’ve done is counted the number of spikes that are involved, and the number seems reasonably normal. You might say that if the number of spikes is the loudness or volume of the code, the volume seems normal. But that doesn’t tell you that the content of the code is normal. We want to look more finely at how the cortex is encoding information to ask whether we can identify deficits in the information content of the code. There may be common deficits across multiple mouse lines or distinct impairments in different genetic forms of autism.
While we focus on neural coding of tactile features, what we’re really interested in are the abstract deficits that are occurring in neural coding in general in the cortex, not specific to the processing of touch.
“There may not be a one-size-fits-all manipulation of inhibition that’s broadly useful here.”
How do your findings inform ideas for treatment?
Honestly, I’m not sure that our study really has a strong prediction for that. There are groups who, going by the original E-I ratio hypothesis, believe that the causative, physiological impairment here is the lack of inhibition. If that model is true, then therapeutic manipulations that restore inhibition should be helpful. For example, there are groups that experimentally use optogenetic or pharmacogenetic approaches to make parvalbumin interneurons more excitable in order to boost inhibition back up again. One study argues that this has some positive effects on social behavior in one of the mouse models4.
It could be that there are some forms of autism in which the decrease in inhibition, which we observed, was actually a direct effect of the gene mutation. And therefore, in those cases, increasing inhibition might actually be useful.
There could be other cases in which the direct effect of the gene mutation was on excitation, and then inhibition adjusted to compensate to restore firing rates. You’d imagine in those cases that the reduction in inhibition was useful to get the brain working again. If you boost inhibition back up, you might actually be undoing that compensation, and that might be harmful in the disease. So I think it just encourages us to think that there may not be a one-size-fits-all manipulation of inhibition that’s broadly useful here.
How do you link these circuit level changes to human data?
We haven’t tried to make a direct link to human cortical physiology here. I think it’s important to do that, but I think we would want to use the mouse work to identify candidate features of circuit function that are impaired and then figure out the best way in humans, maybe with EEG [electroencephalogram] or ECoG [electrocorticography], to see if we can detect signatures of those same impairments.
It sounds like you’re saying animal models might help reveal features of autism.
Examining different genetic models of autism can provide insight about which genetic types of the disorder are similar to each other and which are different from each other. The hope would be that the information would influence clinical evaluations of autism to help better define how we even think about the autism spectrum in humans.
We know from the clinical work that there’s no such thing as one type of autism. We call it a spectrum and whether it’s best thought of as a continuous spectrum, or a collection of 20 disorders that are all different, or a collection of a hundred disorders that are all different remains to be determined.
I’m hopeful that animal models of the disorder will help us figure out how to develop a better understanding of forms of autism in people, including how to group them into subgroups that might suggest effective treatments.
References
- Antoine M.W. et al. Neuron 101, 648-661 (2019) PubMed
- Rubenstein J.L.R. and Merzenich M.M. Genes Brain Behav. 2, 255-267 (2003) PubMed
- Gainey M.A. et al. J. Neurosci. 38, 4749-4761 (2018) PubMed
- Selimbeyoglu A. et al. Sci. Transl. Med. 9, eaah6733 (2017) PubMed
- Sohal V.S. and Rubenstein J.L.R. Mol. Psychiatry Epub ahead of print (2019) PubMed