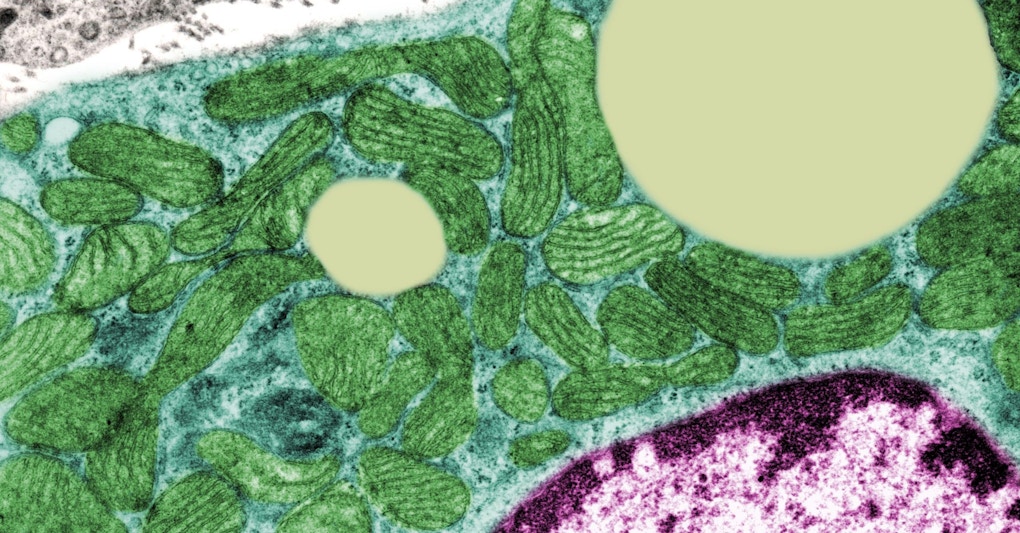
JOSE CALVO / Science Source
The brain uses more energy than any other organ. Fueling this need for energy is mitochondria, the central organelles within cells that convert energy into a form that cells can use. Emerging evidence suggests that mitochondrial dysfunction may play a role in increasing susceptibility to autism spectrum disorder (ASD). To discuss what is known so far about the contribution of mitochondrial dysfunction to the development of ASD, the Simons Foundation Autism Research Initiative (SFARI) hosted a two-day virtual workshop on March 25 and 28, 2022.
Organized by SFARI senior scientist Alan Packer, the workshop featured presentations and discussions by a multidisciplinary group of leading scientists conducting mitochondrial research spanning cellular, molecular, genetic, clinical and pharmacological areas. The goal of the workshop was to review the emerging evidence suggesting that modest deficiencies in mitochondrial function may be an underappreciated risk factor for ASD.
“The success of the field in associating nuclear genes with ASD has perhaps overshadowed the possible role of mitochondrial genetic variation, which we wanted to explore in some depth in this workshop,” says Packer.
Mitochondrial genome analysis in ASD
Day 1 of the workshop featured six presentations covering genetic, molecular, and cellular consequences of mitochondrial dysfunction and their relevance for neurodevelopmental conditions. In the first talk, SFARI Investigator Zhenglong Gu of Fudan University, China began by asking, why focus on mitochondria? Mitochondria are a cellular furnace supporting brain function. They generate energy in the form of adenosine triphosphate (ATP) molecules through oxidative phosphorylation (OXPHOS) not only for cellular tasks, but also for many critical cellular processes, including epigenetic regulation, inflammation, programmed cell death and calcium signaling.
Although most DNA is packaged within the nucleus of the cell, mitochondria also have their own DNA, known as mitochondrial DNA (mtDNA). Especially relevant in the context of disease is the fact that mtDNA can mutate at a much higher rate compared to the nuclear genome. Gu discussed how mutations in mtDNA are implicated in the risk of disease both during aging and during development. One unique characteristic of mtDNA is that typical and mutated mtDNA can co-exist within an individual — a phenomenon known as heteroplasmy, which has clinical relevance to numerous conditions. But large-scale studies of mtDNA heteroplasmies are a challenge because there are not as many available tools to study mtDNA as there are for nuclear DNA. Gu and his team set out to tackle this challenge and ultimately developed a new human mtDNA sequencing method with a simplified experimental workflow, low cost, low error rate and high power for detecting mtDNA heteroplasmy1. This new method will be useful for studies of single cells as well as for clinical and epidemiological studies of human populations with diseases.
One possible cause of ASD relates to the variation in mtDNA. In a large genomic study of ASD2, Gu and his team looked at mtDNA sequences from children with ASD, along with their mothers and unaffected siblings, from the Simons Simplex Collection. In these families, only one child in the family has ASD, and the overall familial risk for ASD is low. The results showed higher frequencies of pathogenic mtDNA mutations in children with ASD compared to their unaffected siblings, which was found to stem from a unique mother-to-child transmission pattern found for children with ASD, where mtDNA heteroplasmies of high pathogenic potential are passed down. He plans to further explore the functional consequences of mtDNA heteroplasmy on physiology and health in newborns and children, and ultimately, how to address those mutations.
In agreement with Gu, Nicole Lake of Yale University stressed the need for development of new tools for mitochondrial genome analyses. Understanding which mtDNA variants contribute to disease is difficult. For the mitochondrial genome, this is compounded by the limitations of most computer-based tools for genetic analyses, which are optimized for the nuclear genome. Although genomic databases are useful for studying the contribution of gene mutations to ASD, these databases have focused on nuclear DNA and ignored mtDNA. In her talk, Lake discussed how she and her colleagues leveraged the sequencing data available in one such database, the Genome Aggregation Database (gnomAD), to build a new mtDNA population database.
Lake described several filters that they applied to gnomAD samples (76,156 samples), which were designed to address technical challenges, including those related to the quality of samples and the detection of heteroplasmy. The final gnomAD mitochondrial call set (56,434 samples) included nearly 11,000 unique variants at more than half of all DNA bases in the mitochondrial genome, with about 15 percent of the variants being heteroplasmic3. The gnomAD mtDNA database provides new tools for mtDNA analysis for disease studies, including for ASD research, and is freely available on the gnomAD browser. Lake also pointed out another resource for mitochondrial research in ASD, the SFARI Genomes Browser, developed by SFARI Investigator Monkol Lek in collaboration with SFARI director of data and analytics Natalia Volfovsky. It currently contains mtDNA variant calls from approximately 2,500 genomes from SPARK, and these have been processed using the gnomAD pipeline as well.
Shared mitochondrial pathways of ASD risk genes
Hundreds of risk genes have been identified for ASD. Karun Singh of the University of Toronto raised two questions that research on the genetics of ASD has been struggling to answer: “Are there common pathways between these genes? And how can we organize them into something more understandable than just hundreds of independent risk genes?” To answer these questions, Singh and his team explored which ASD risk genes interact with each other or converge into common signaling networks at the protein level. Most ASD risk genes encode proteins, and protein-protein interaction (PPI) is an important mechanism of signaling in the brain. Singh identified a shared PPI network map and common pathways of 41 ASD risk genes, using BiolD, a tool for proximity-based protein labeling that Singh is specifically using in neurons4.
Singh’s analysis revealed that one of the most significant pathways shared in the 41 ASD risk gene PPI network involve synaptic transmission, supporting one leading hypothesis in ASD research that implicates altered functioning of synapses in the condition. More surprising to Singh, however, was the finding that about two-thirds of them interact with tricarboxylic acid (TCA) and mitochondrial pathways4. They further explored some of these PPI network genes not previously associated with mitochondrial and metabolic processes in the brain, namely, TAOK2 and SYNGAP1. The absence of each gene resulted in altered cellular respiration in cortical neurons to varying degrees4. This suggested that a subset of ASD risk genes have either direct or indirect effects on mitochondrial function in the brain, and there may be many more that have yet to be investigated. Singh ended his talk with a question related to timing: if ASD risk genes are impacting mitochondrial function, when do they do this during neurodevelopment?
Mitochondrial involvement in neuron fate acquisition and neuronal development
The timing of neuronal development has been a longstanding focus of Pierre Vanderhaeghen of KU Leuven. In his talk, Vanderhaeghen pointed out that neurogenesis in the human cortex is much more prolonged (4 months) than in the mouse cortex (7 days), for which there may be a genetic mechanism. Similarly, neuronal maturation is considerably extended in the human cortex, taking up to two decades in the prefrontal cortex, while it takes one to two months in the mouse cortex. Thus, understanding the links between brain evolution and brain developmental timing could provide hints about neurodevelopmental conditions including ASD.
One possibility is that species-specific changes in metabolism (and mitochondria) could also have an impact on neuronal developmental timing, a hypothesis that Vanderhaeghen and his team turned their attention to in studies of mitochondrial dynamics during neurogenesis in the mouse and human cortex. By looking at the stem cells of early brain development, which can either generate differentiating neurons or self-renew to give rise to more of themselves, the researchers showed that decisions about the future identity of the cell (cell fate) involves the mitochondria5. Specifically, cells with fragmented mitochondria undergoing fission after mitosis were more likely to become neurons, whereas cells undergoing mitochondrial fusion self-renewed to continue being stem cells.
When Vanderhaeghen and his team changed this pattern of mitochondrial fission and fusion shortly after mitosis, they were able to switch the fate of the cells5. Typically, it has been thought that the decision to become a neuron or a stem cell is made prior to mitosis. Vanderhaeghen showed that the decision can still be made after mitosis, indicating a new period of cell fate plasticity after mitosis. And this period is double the time in humans versus in mice. In essence, the human cells have twice as much time as mouse cells to switch their cell fate, which could contribute to species-specific developmental timing.
To explore whether mitochondria influence these species-specific features of cortical neuron maturation, Vanderhaeghen and his team showed that the development of mitochondria (size and quantity) is slower, and mitochondrial metabolic activity is lower, during the maturation of neurons in the human cortex than in the mouse cortex6. Vanderhaeghen also discussed his other recent experiments showing that boosting mitochondrial metabolic activity in developing neurons resulted in an accelerated rate of maturation6, pointing to a causal role of mitochondria in the timing of neuronal maturation. This work indicates that mitochondria are regulators of the timing of species-specific neuronal development.
In the discussion, Packer pointed out the relevance of Vanderhaeghen’s work to ASD, in that a number of other recent studies have suggested that mutations in ASD risk genes can have significant effects on the timing of neuronal differentiation in mouse and human cell models. Vanderhaeghen acknowledged the possibility of there being a disruption or acceleration of developmental timing in ASD and a potential link with mitochondrial function.
In agreement with Vanderhaeghen and Singh, SFARI Investigator Elizabeth Jonas of Yale University stressed that understanding how cell fate and neuronal development are affected at different stages of brain development is very important for understanding ASD. Mitochondrial changes in some disorders may be developmentally regulated in terms of timing. Over many years, Jonas and her research team have done studies testing the hypothesis that, during early development, an opening in a leak channel that regulates inner mitochondrial membrane ATP production efficiency creates an immature type of metabolism in which ATP is made from aerobic glycolysis rather than from mitochondria. In a later developmental stage, this ATP synthase leak channel closes in response to synaptic and other stimuli, and the mitochondria start making ATP — essentially shifting to a mature, more efficient oxidative metabolism. According to Jonas, the gradual shift from an immature glycolytic metabolism to mature oxidative metabolism in the synapse or neuron in different cell types and brain regions should occur at key developmental time points, otherwise the neural wiring may develop abnormally.
As one example, Jonas discussed her findings of a mitochondrial inner membrane leak in fragile X syndrome (FXS)7, a leading genetic cause of ASD. In fibroblasts from people with FXS and in a mouse model of FXS, a lack of the FMRP gene caused a persistent glycolytic leak metabolism. Closure of the leak allowed the maturation of synapses and normalized certain ASD-like behaviors.
Complexity and variety in phenotypes of mitochondrial dysfunction
Cecilia Giulivi of the University of California, Davis, noted that many different models of ASD point to mitochondrial dysfunction, but not all mitochondrial dysfunction is the same. The phenotypes of mitochondrial dysfunction are complex and vary across models and affected individuals, with mitochondrial dysfunction being a cause or a contribution to morbidity. Because of the complexity and variety in mitochondrial phenotypes, there are currently no standardized one-size-fits-all diagnostic or testing approaches for mitochondrial dysfunction that can be applied to all potential patients.
Because mitochondrial function is not the same in all tissues, Giulivi and her collaborators collect a wide array of samples, including from the brain and from peripheral samples (e.g., peripheral blood mononuclear cells, plasma, cord blood, placenta, amniocytes, breast milk, skin fibroblasts). Giulivi’s research shows the value of these peripheral markers of mitochondrial dysfunction in reflecting conditions at the level of the brain8.
Giulivi also discussed her past SFARI-funded research exploring gene-environment interactions in ASD, with a focus on exposure to environmental triggers after birth or on fetal imprinting during pregnancy. She and her team have examined exposure to the widely used flame retardant BDE-49, a type of polybrominated diphenyl ether (PBDE), which has been steadily increasing in the environment over the past few decades. They found that changes in brain mitochondria in ASD are worsened following PBDE exposure9. Further, this PBDE-mediated mitochondrial dysfunction was enhanced by a genetic background associated with ASD (deficiency of PTEN, a negative modulator of the PI3K/Akt pathway). Giulivi’s findings on genetic backgrounds that confer susceptibility to environmental exposures have important implications for the energy balance of the brain during development.
Mitochondrial effects on social behavior
Day 2 of the workshop featured another six presentations, which covered the impact of mitochondrial deficiencies on specific experimental systems including animal and stem cell models of neurodevelopmental conditions, as well as in affected individuals. Also covered were clinical profiles of mitochondrial deficiency, as well as therapeutic approaches for mitochondrial diseases that might be relevant for ASD.
Many individuals with fragile X syndrome, which is caused by FMR1 mutations, also have ASD. In her talk, Claudia Bagni of the University of Lausanne described how FMRP represses mRNA translation and regulates synaptic plasticity in multiple ways, including by interacting with the CYFIP1 gene10. Mutations in CYFIP1 have been associated with 15q11.2 BP1-BP2 deletion/duplication syndrome, and 30 percent of these individuals also have ASD11,12.
Bagni discussed her work on the contributions of CYFIP1 mutations to human disease, using a mouse model of CYFIP1 haploinsufficiency13. In this model, she showed findings of compromised brain functional connectivity and ASD-like behaviors, such as changes in motor coordination, sensorimotor gating and sensory perception13, as well as preliminary data on a decrease in measures of social interaction.
To explore the molecular mechanisms for these changes, Bagni and her team used as an experimental model the fruit fly Drosophila melanogaster. Drosophila genome is 60 percent homologous to that of humans and about 75 percent of the genes responsible for human diseases have homologs in flies. Bagni and her team found that flies with CYFIP haploinsufficiency displayed deficits in ASD-relevant social behaviors, as well as brain mitochondrial hyperactivity14. She uncovered a key molecular mechanism for this — in the flies with CYFIP insufficiency, the mitochondrial transporter protein Aralar shuttles the main inhibitory neurotransmitter gamma aminobutyric acid (GABA) from the synapse to the mitochondria, ultimately leading to decreased GABA transmission and increased mitochondrial activity. Either enhancing GABA levels or inhibiting mitochondrial hyperactivity improved social behavior in the mutant flies. As aralar is also expressed in mammals (SLC25A12), this mechanism offers insight into new potential targets to improve social deficits and GABA dysfunctions in more complex systems.
Variation in mitochondrial DNA mutations
In the next talk, Douglas Wallace of Children’s Hospital of Philadelphia discussed the observation that mitochondrial dysfunction can lead to an array of different clinical phenotypes, depending on the level of mitochondrial energy deficiency. For example, the tRNALeu(UUR) np m.3243A>G mutation at certain heteroplasmic levels may lead to ASD. Individuals with 10 to 30 percent of the mutation manifest ASD or diabetes, while individuals with 70 percent of the mutation may present with hypertrophic cardiomyopathy and myopathy of the MELAS (mitochondrial encephalomyopathy, lactic acidosis and stroke-like episodes) syndrome, and those with 100 percent of the mutation have lethal childhood disease.
To address the question of how the same mutation can yield such different phenotypes, he prepared cells with the same nucleus but different percentages of the m.3243A versus G mutation ranging from 0 to 100 percent. Analysis of the nuclear gene expression of the cells with different 3243G heteroplasmy levels revealed that the different mtDNA heteroplasmic genotypes correlated with discrete nuclear gene expression patterns and that these changes in gene expression coordinately affected known certain ASD-associated genes.
As proof that mild mitochondrial dysfunction is sufficient to cause ASD, Wallace reported the creation of a mouse harboring a single mitochondrial DNA (mtDNA) nucleotide change that cause a 60 percent reduction in the activity of the first mitochondrial oxidative phosphorylation enzyme, NADH dehydrogenase (ND6P25L), also known as complex I. This mouse manifested traits related to ASD. Hence, a partial mitochondrial defect is sufficient in itself to cause ASD. Wallace concluded that differences in energy deficiency may be important in the etiology of a variety of neurological and neuropsychiatric phenotypes, including ASD.
In the discussion, the question was raised, what determines whether an individual with a mitochondrial change has ASD versus depression versus another neuropsychiatric disorder? Wallace responded that the degree of mitochondrial respiratory dysfunction is likely relevant, as is the relative amount of mitochondrial reactive oxygen species production, as well as the mitochondrial metabolites which alter nuclear gene expression. Only certain combinations of mitochondrial dysfunction may generate a particular neuropsychiatric phenotype.
Neuronal network changes in mitochondrial disorders
In the next talk, SFARI Investigator Nael Nadif Kasri of the Radboud University Medical Centre reiterated that the proportion of mutated mtDNA needs to exceed a certain critical threshold for symptoms to arise, and that the percentage of mutated mtDNA varies between individuals as well as among organs and tissues within the same individual (mtDNA heteroplasmy). In collaboration with Tamas Kozicz of the Mayo Clinic, Kasri uses human induced pluripotent stem cells (iPSCs) to model the neurodevelopmental aspects of cells with different levels of heteroplasmy. These iPSCs are derived from individuals with MELAS (mitochondrial encephalomyopathy, lactic acidosis, and stroke-like episodes), a multisystem mitochondrial disease known to co-exist with ASD and neuropsychiatric symptoms. MELAS is caused in most cases by an m.3243A>G pathogenic variant in the MT-TL1 gene.
Kasri discussed the work that he and Kozicz do with iPSCs from MELAS patients with this mutation. These iPSCs are differentiated into excitatory neurons with typical (low heteroplasmy) and atypical (high heteroplasmy) mitochondrial function. In neurons with high heteroplasmy, they found not only mitochondrial dysfunction (e.g., defects in mitochondrial respiration and abundance), but also that the dysfunction of mitochondria affected the structure and function of neurons, as shown by less complex dendrites, fewer excitatory synapses and less synaptic and network activity15. These changes in energy metabolism and neuronal networks could potentially explain the neuropsychiatric manifestations of MELAS and other mitochondrial diseases.
In another study aiming to get a better sense of how the m.3243A>G mutation in MELAS affects the brain16, Kasri and colleagues found that the expression of many genes linked to mitochondrial respiration and synaptic function was affected in the iPSC-derived neurons with high levels of m.3243A>G heteroplasmy. And they were able to partially rescue these changes in gene expression and neuronal network activity using sonlicromanol, a clinical stage II drug that targets mitochondrial function.
Kasri raised the question, Can we identify specific neuronal network phenotypes that are specific to ASD? And are they associated with mitochondrial dysfunction? He summarized a large body of his SFARI-funded work15, 17–19 in which neuronal network phenotypes were shown to be specific for genes linked to syndromes that affect neurodevelopment, such as MELAS, Dravet syndrome and Kleefstra syndrome.
Mitochondrial causes of neuropsychiatric symptoms
In the next talk, Stewart Anderson of the Children’s Hospital of Philadelphia discussed some of his work on 22q11.2 deletion syndrome (22q11DS), a condition in which a small part of chromosome 22 is missing. About 40 percent of children with 22q1DS have ASD, and 20–30 percent later develop schizophrenia. Anderson explained that there is a lot of overlap in the features and risk genes of schizophrenia and ASD.
Six of the deleted genes in the 22q11.2 region encode proteins that localize to mitochondria. To explore mitochondrial changes in 22q11DS (in collaboration with George Wallace), he used excitatory neurons differentiated from the iPSCs of individuals from three groups: 22q11DS with schizophrenia, 22q11DS without schizophrenia and neurotypicals20. Those with 22q11DS and schizophrenia had lower levels of ATP compared not only to neurotypicals but also to the 22q11DS group without schizophrenia. The same pattern was observed with lymphoblastoid cell lines (LCLs), in which there was decreased mitochondrial biogenesis in LCLs from 22q11DS individuals with schizophrenia compared to those with 22q11DS without schizophrenia. The differences between people with 22q11DS with and without schizophrenia led them to hypothesize that there may be a compensatory phenomenon in the latter group that protects them from developing schizophrenia.
Using a “chicken or the egg” analogy, Anderson raised the question, Are the mitochondria in the LCLs of individuals with 22qDS changed as a result of having schizophrenia? Or are mitochondrial changes in LCLs from people with 22qDS occurring prior to the development of schizophrenia? In the instance of ASD, many children with the condition generally are not typical in the areas of sleeping, eating or exercise, all of which affect mitochondrial and metabolic function. This makes it unclear whether mitochondrial changes are upstream or downstream of changes in eating, sleeping and exercise. Anderson’s current work is exploring answers to these questions, including whether metabolic deficits in LCLs could be a useful biomarker for schizophrenia risk in the context of 22qDS.
Environment, mitochondria, and ASD
While most of the workshop presentations highlighted observations of mitochondrial deficiencies, Richard Frye of the Phoenix Children’s Hospital pointed out observations of mitochondrial overactivity in ASD, particularly in a subset of children with developmental regression21. He described one of his studies showing that children with ASD plus developmental regression had higher maximal respiratory capacity and reserve capacity — which is essentially a measure of the health of the mitochondria — than the ASD group without developmental regression, or than children without ASD22. Because children with ASD generally show indicators of high oxidative stress and free radicals, Frye postulated that the mitochondria in children with developmental regression may be trying to compensate for high levels of oxidative stress. Further, there may be interactions of mitochondria under stress with high levels of free radicals, genetic variation and exposures to environmental toxins, resulting in the mitochondria trying to compensate for these deficits.
Given the possible subsets of ASD with different gene variants, causes and history of regression, Frye decided to cluster LCLs of children with ASD into different groups based on the baseline reserve capacity of their mitochondria. This revealed two distinct groups of LCLs in children with ASD. One group of children with ASD had a reserve capacity that was similar to neurotypical children, both at baseline and when stressed (after adding DMNQ, an inducer of oxidative stress). The other group with ASD had a higher reserve capacity at baseline but a greater loss of reserve capacity when stressed, suggesting a greater sensitivity to oxidative stress. A closer look at the mitochondrial respiratory function indicated that what may distinguish LCLs in ASD from unaffected LCLs is not higher levels of oxidative stress per se, but how the mitochondria can handle oxidative stress23. In the LCLs with atypical reserve capacity, Frye also found that prolonged exposure to environmental agents (for example, exposure to reactive oxygen species) increased baseline mitochondrial respiration and sensitivity to oxidative stress, pointing to interactions with environmental factors which may induce this mitochondrial abnormality.
Mitochondria are highly sensitive to environmental agents. Frye discussed his work showing the effects of prenatal environmental influences on long-term changes in mitochondrial respiration. He looked at environmental influences from two perspectives: (1) exposure to air pollution during the prenatal period24 and (2) prenatal exposure to nutritional metals and early life exposure to toxic metals25. Both were highly correlated with mitochondrial dysfunction in childhood, especially in children with ASD who had developmental regression. Frye suggested that prenatal and early-life exposure to environmental agents disrupts neurodevelopment, particularly in the ASD subtype with developmental regression, through mechanisms including long-term changes in mitochondrial respiration.
Emerging therapies
Mitochondrial diseases are difficult to treat because of their biochemical, genetic and clinical complexity. In her talk, Shamima Rahman of University College London discussed how the field of mitochondrial research is progressing rapidly, with increasing number of genes being uncovered, but the challenges of developing effective therapies in the context of this complexity remain. Although curative therapies do not yet exist for the vast majority of children with primary mitochondrial diseases, there have been advances in the development of therapies in preclinical and early-phase human interventional studies, with some of these candidate therapies being potentially relevant for ASD.
Rahman described approaches to treating primary mitochondrial diseases that are considered to be disease agnostic, that is, they are generic approaches that are not specific to a particular disease and could therefore be relevant for ASD treatment. She focused on four of these approaches: (1) targeting reactive oxygen species, (2) harnessing mitochondrial biogenesis, (3) stabilizing the mitochondrial membrane and (4) targeting mitophagy through inhibition of the mammalian target of rapamycin (mTOR) pathway. She talked about specific compounds being planned or developed within each of these four approaches.
With respect to the antioxidant approach targeting reactive oxygen species, Rahman mentioned that phase 2b/3 trials of the mitochondrial redox modulators PTC743 and KH176 (sonlicromanol) are currently in progress for treatment of primary mitochondrial disease in children (https://clinicaltrials.gov/ct2/show/NCT04378075; https://www.clinicaltrials.gov/ct2/show/NCT04846036). The second approach — harnessing mitochondrial biogenesis — has not been without challenges. Although Rahman showed promising data from human patient cell models26, she also acknowledged the mixed findings from animals models as well as from human studies of bezafibrate27, resveratrol28, and niacin29 in mitochondrial disease.
The third pharmacologic approach that Rahman discussed involves stabilization of the mitochondrial membrane, particularly by targeting cardiolipin, an important phospholipid in the inner mitochondrial membrane. Elamipretide (a tetrapeptide that targets cardiolipin) has been shown in a phase I/II trial to increase mitochondrial respiration and improve a measure of physical fatigue in patients with primary mitochondrial disease30. But, in a subsequent phase III trial, this drug had mixed results between subgroups of patients with mitochondrial disease, underscoring the challenges involved in therapy development for diseases that are as complex and heterogeneous as mitochondrial diseases.
The fourth pharmacologic approach — targeting mitophagy through mTOR inhibition — aims to enhance mitochondrial translation, reduce mitophagy and increase the synthesis of nucleotides, glucose and lipids. However, mTORC1 inhibition has so far yielded mixed results in mouse models of mitochondrial disease31,32 as well as in children with mitochondrial diseases33.
Rahman ended her talk by discussing the multistep process involved in bridging the translational gap, starting from natural history studies, to biomarkers and outcome measures, to candidate therapies and, finally, to clinical trials. Thanks to this process, a large number of trials for primary mitochondrial disease are currently in progress. Future studies will start to answer remaining questions such as: Which treatment is helpful for which patient group? Which outcome measures are best? And which, if any, of these approaches are applicable to ASD treatment?
Next steps
An overall concluding discussion was led by Packer and Kelsey Martin director of SFARI and the Simons Foundation Neuroscience Collaborations. SFARI has developed a number of resources over the past decade for the scientific community, including ASD cohorts, stem cell as well as animal models (mice, rats, zebrafish) to study different aspects of ASD biology. Given the availability of these resources, Packer asked the workshop attendees for their thoughts on future key areas of study that would further test the idea that mitochondrial dysfunction might contribute to the etiology of ASD.
Wallace responded that one area requiring further exploration is determining how common mitochondrial dysfunction is in many of the classical nuclear ASD risk genes. Comparing mtDNA variation to nuclear DNA variation in common mitochondrial dysfunction may be useful, and a battery of tests would be needed to assess a variety of aspects of mitochondrial function in order to assess how important mitochondria are in the development of ASD or any other neuropsychiatric disorder.
Martin stimulated discussion regarding the level of resolution where key differences could be detected. Wallace noted that, in ASD, changes could be mitochondrial, tissue or cell specific. Mitochondria regulate all of metabolism and could encompass hundreds of ASD risk genes at many levels. Giulivi concurred that there are many different mechanisms driven by different genes leading to mitochondrial dysfunction. Bagni emphasized a focus on two main compartments, namely, the cell body and synapse, and fine-tuning the molecules/modulators of mitochondrial activity would be the next step.
Finally, a critical point raised by Kozicz is the degree to which environmental factors play a role in the precipitation of various neurodevelopmental disorders. It remains unclear whether mitochondrial dysfunction, even considering various levels of energy deficiency in the brain, would be sufficient to cause ASD. An important area of future study would be to determine which subsets of the population with ASD may have mitochondrial dysfunction as the primary cause of their neurodevelopmental condition.
“This was an incredibly stimulating discussion on a topic that is still relatively understudied in autism research,” said Martin. “We have learned a lot and will leverage this knowledge to better understand how SFARI can contribute to advance research in this space.”
References
- Guo X. et al. NAR Genom. Bioinform. 2, lqaa065 (2020) PubMed
- Wang Y. et al. PLoS Genet. 12, e1006391 (2016) PubMed
- Laricchia K.M. et al. Genome Res. 32, 569-582 (2022) PubMed
- Murtaza N. et al. bioRxiv (2022) Preprint
- Iwata R. et al. Science 369, 858-862 (2020) PubMed
- Iwata R. et al. bioRxiv (2021) Preprint
- Licznerski P. et al. Cell 182, 1170-1185 (2020) PubMed
- Wang J. et al. Int. J. Mol. Sci. 22, 9171 (2021) PubMed
- Napoli E. et al. Toxicol. Sci. 132, 196-210 (2013) PubMed
- De Rubeis S., et al. Neuron 79, 1169-1182 (2013) PubMed
- Cox D.M. et al. Int. J. Mol. Sci. 16, 4068-4082 (2015) PubMed
- SFARI Gene – Welcome. SFARI Gene. Accessed April 1, 2022. https://gene.sfari.org/
- Domínguez-Iturza N. et al. Nat. Commun. 10, 3454 (2019) PubMed
- Kanellopoulos A.K. et al. Cell 180, 1178-1197 (2020) PubMed
- Klein Gunnewiek T.M. et al. Cell Rep. 31, 107538 (2020) PubMed
- Klein Gunnewiek T.M. et al. Stem Cell Reports 16, 2197-2212 (2021) PubMed
- Frega M. et al. Nat. Commun. 10, 4928 (2019) PubMed
- Frega M. et al. Cell Rep. 30, 173-186 (2020) PubMed
- Linda K. et al. Autophagy 18, 423-442 (2022) PubMed
- Li J. et al. JAMA Psychiatry 78, 911-92 (2021) PubMed
- Frye R.E., et al. J. Pediatr. Neurol. 9, 427-434 (2011) Article
- Singh K. et al. Ann. Clin. Transl. Neurol. 7, 683-694 (2020) PubMed
- Rose S. et al. FASEB J. 31, 904-909 (2017) PubMed
- Frye R.E. et al. Mol. Psychiatry 26, 1561-1577 (2021) PubMed
- Frye R.E. et al. Transl. Psychiatry 10, 223 (2020) PubMed–
- Kanabus M. et al. J. Inherit. Metab. Dis. 39, 415-426 (2016) PubMed
- Steele H. et al. EMBO Mol. Med. 12, e11589 (2020) PubMed
- Løkken N. et al. J. Inherit. Metab. Dis. 44, 1186-1198 (2021) PubMed
- Pirinen E. et al. Cell Metab. 31, 1078-1090 (2020) PubMed
- Karaa A. et al. Neurology 90, e1212-e1221 (2018) PubMed
- Johnson S.C. et al. Science 342, 1524-1528 (2013) PubMed
- Siegmund S.E. et al. Hum. Mol. Genet. 26, 4588-4605 (2017) PubMed
- Sage-Schwaede A. et al. Ann. Clin. Transl. Neurol. 6, 1877-1881 (2019) PubMed